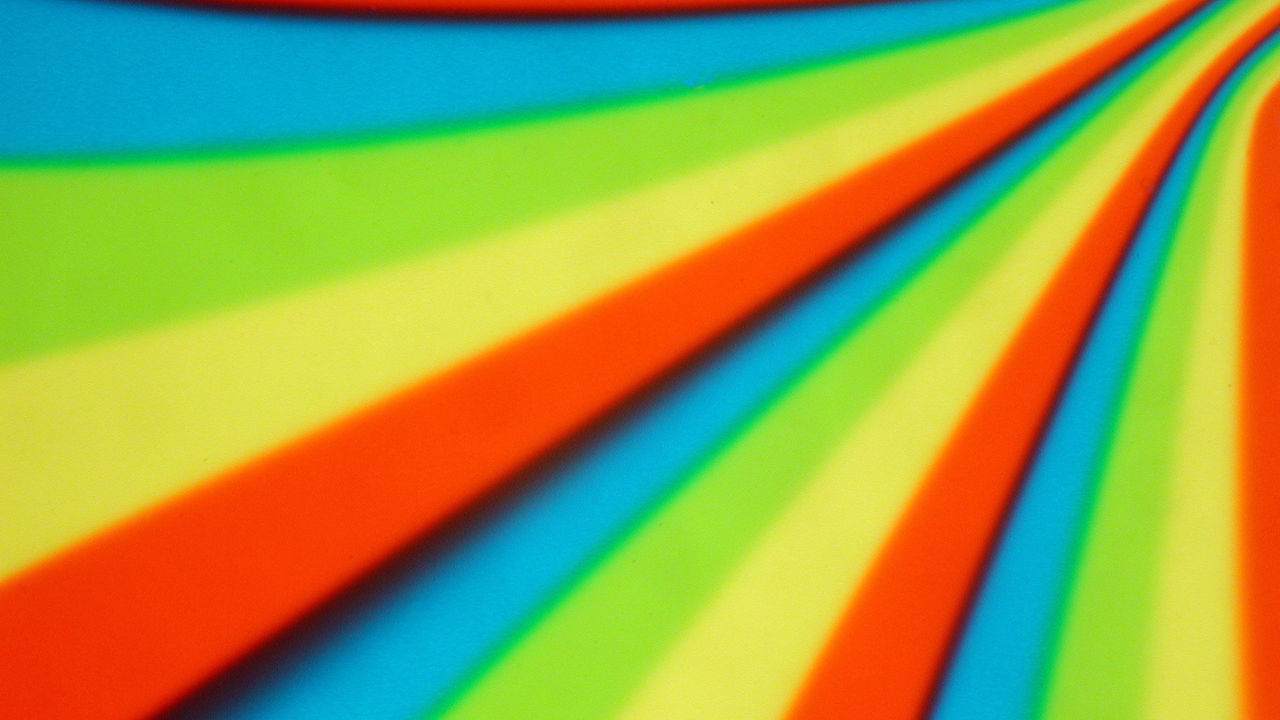

Pronounced Folk :-)
Microtechnology Projects
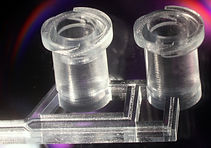
3D-Printing
The “3D-printing revolution” is now embracing the range of feature sizes and materials that are starting to appeal to microfluidic engineers and users. There are multiple features of 3D-printing technology that make it very attractive when compared to PDMS or plastic molding. Using a simple desktop computer and prior to fabrication, the design is digitally built in all 3D detail as a 3D CAD file, can be assembled in modules by remotely collaborating teams, digitally inspected in 3D, and the mechanical and fluidic behavior of the device can be inexpensively simulated using finite-element modeling. Structures are created by adding materials, without requiring any etching or dissolution, so processing is environmentally and economically efficient. By contrast, PDMS and plastic molding are limited to layered designs, where each layer must be processed separately and bonded; knowledge of the final 3D structures prior to fabrication is not realistic and collaborative design between different teams is impractical. Interestingly, some 3D-printing approaches are now becoming more affordable than PDMS molding due to the availability of low-cost desktop printers and supplies. Last but not least, the inherently low throughput of PDMS molding and the high-cost of plastic molding have made it difficult to launch microfluidic device companies; 3D printing technologies offer a natural path from prototype to commercialization that should engage many ongoing translational efforts in microfluidics. In biomedicine, shortening the time from prototype to product enables personalized devices, accelerates R&D, and helps reduce the cost of access to healthcare. Thus, we predict that in the next few years most PDMS and plastic molding activities in academia will have been replaced by 3D-printing.
References:
A Naderi, N Bhattacharjee, A Folch, "Digital Manufacturing for Microfluidics", Annual review of biomedical engineering 21: 325-36 (2019).
AP Kuo, N Bhattacharjee, YS Lee, K Castro, YT Kim, A Folch, "High‐Precision Stereolithography of Biomicrofluidic Devices", Advanced Materials Technologies 4: 1800395 (2019).
YT Kim, S Bohjanen, N Bhattacharjee, A Folch, "Partitioning of hydrogels in 3d-printed microchannels", Lab on a Chip 19: 3086-3093 (2019).
YS Lee, N Bhattacharjee, A Folch, "3D-printed Quake-style microvalves and micropumps", Lab on a Chip 18: 1207-1214 (2018).
Y.T. Kim, K. Castro, and A. Folch, “Digital Manufacturing of Selective Porous Barriers in Microchannels Using Multi-Material Stereolithography”, Micromachines 9: 125 (2018).
N. Bhattacharjee, C. Parra-Cabrera, Y.T. Kim, A.P. Kuo, and A. Folch, “Desktop-Stereolithography 3D-Printing of a Poly(dimethylsiloxane)-based Material with Sylgard-184 Properties, Advanced Materials, 1800001 (2018).
AK Au, W Huynh, LF Horowitz, A Folch, "3D‐printed microfluidics", Angewandte Chemie International Edition 55: 3862-3881 (2016).
A. Urrios, C. Parra-Cabrera, A. M. Gonzalez-Suarez, N. Bhattacharjee, L. G. Rigat-Brugarolas, U. Nallapatti, J. Samitier, C. A. DeForest, F. Posas, J. L. Garcia-Cordero, and A. Folch, “3D-Printing of transparent bio-microfluidic devices in PEG-DA”, Lab on a Chip 16: 2287 (2016).
N Bhattacharjee, A Urrios, S Kang, A Folch, "The upcoming 3D-printing revolution in microfluidics", Lab on a Chip 16: 1720-1742 (2016). [COVER]
A.K. Au, N. Bhattacharjee, L. Horowitz, T. Chang, and A. Folch, “3D-printed microfluidic automation”, Lab on a Chip 15: 1934 (2015).
W. Lee, D. Kwon, B. Chung, G.Y. Jung, A. Au, A. Folch, and S. Jeon, "Ultrarapid detection of pathogenic bacteria using a 3D immunomagnetic flow assay", Anal. Chem. 86: 6683 (2014).
A.K. Au, W. Lee, and A. Folch, "Mail-order microfluidics: evaluation of stereolithography for the production of microfluidic devices", Lab on a Chip 14: 1294 (2014).
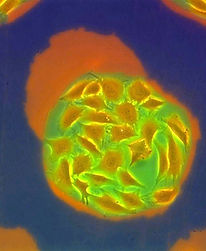
Cellular Micropatterns
Tissue function is modulated by the spatial organization of cells on a sub-millimeter scale. For this reason, artificial replication of cellular microstructures is important in understanding, measuring, and simulating their in vivo functions in the laboratory. We are continuously developing techniques that allow us to selectively attach cells on a variety of substrates, including biocompatible polymers, hydrogels, and porous substrates.
References:
J.W. Cheng, C.G. Sip, P.R. Lindstedt, R. Boitano, B.M. Bluestein, L.J. Gamble, and A. Folch, “'Chip-on-a-Transwell' Devices for User-Friendly Control of the Microenvironment of Cultured Cells”, ACS Applied Bio Materials 2: 4998 (2019).
Ellen Tenstad, Anna Tourovskaia, A. Folch, Ola Myklebost, and Edith Rian, “Extensive adipogenic and osteogenic differentiation of patterned human mesenchymal stem cells in a microfluidic device”, Lab Chip 10: 1401 (2010).
Rettig, J. R. and Folch, A. “Large-Scale Single-Cell Trapping and Imaging Using Microwell Arrays”, Analytical Chemistry 77: 5628-5634 (2005).
Tourovskaia, A., Figueroa-Masot, X. and Folch, A., “Differentiation-on-a-chip: A Microfluidic Platform for Long-Term Cell Culture Studies”, Lab on a Chip 5:14 (2005).
Li, N., Tourovskaia, A., and Folch, A. “Biology on a Chip: Microfabrication in Cell Culture Studies”, Critical Reviews in Biomedical Engineering 31:68 (2003).
Tourovskaia, A., Barber, T., Wickes, B., Hirdes, D., Grin, B., Castner, D. G., Healy, K. E., and Folch, A. “Micropatterns of Chemisorbed Cell Adhesion-Repellent Films Using Oxygen Plasma Etching and Elastomeric Masks”, Langmuir 19:4754 (2002).
Folch, A. and Toner, M. “Microengineering of Cellular Interactions”, Annual Rev. of Biomedical Engineering 2: 227 (2000).

Microfluidic automation
With a few exceptions, microfluidic devices are notoriously difficult to use. At best, they are based on non-intuitive user interfaces and complex tubing setups that are prone to bubble nucleation and human error. We believe that, in order to become a standard technology in clinical devices, microfluidic automation should draw some inspiration from car automation standards, whereby the user operates an intuitive user interface that commands a computer (hidden from the user), which in turns drives a car -- the user no longer drives the car. We are developing microfluidic systems where all the layers of automation (microvalves and micropumps) are hidden from the user, they can be programmed via a battery-operated mini-processor, and the user can introduce all the fluids into the device via intuitive interfaces such as a multi-well plate and standardized connectors.
References:
Adina Scott, Anthony K. Au, Elise Vinckenbosch, and Albert Folch, “A microfluidic D-subminiature connector”, accepted to Lab on a Chip (2013).
A. K. Au, H. Lai, B. R. Utela, and A. Folch, “Microvalves and Micropumps for BioMEMS”, Micromachines 2, 179 (2011).
H. Lai and A. Folch, "Design and characterization of "single-stroke" peristaltic PDMS micropumps", Lab Chip 11, 336 (2011).
J. M. Hoffman, M. Ebara, J. J. Lai, A. S. Hoffman, A. Folch, and P. Stayton, "A helical flow, circular microreactor for separating and enriching smart polymer-antibody capture reagents", Lab Chip 10, 3130 (2010).
Lam, E.W., Cooksey, G.A., Finlayson, B.A., and Folch, A. “Microfluidic Circuits with Tunable Flow Resistances”, Appl. Phys. Lett. 89: 164105 (2006).
Hsu, C.-H. and Folch, A. “Spatiotemporally-Complex Concentration Profiles Using a Tunable Chaotic Micromixer”, Appl. Phys. Lett. 89: 144102 (2006).
Frevert, C.W., Boggy, G., Keenan, T.M., and Folch, A. “Measurement of cell migration in response to an evolving radial chemokine gradient triggered by a microvalve”, Lab on a Chip 6: 849 (2006).
Li, N., Hsu, C.-H., and Folch, A. “Parallel mixing of Photolithographically-Defined Nanoliter Volumes Using Elastomeric Microvalve Arrays”, Electrophoresis 26: 3758-3764 (2005).
Hsu, C.-H. and Folch, A. “Microfluidic Devices with Tunable Microtopographies”, Applied Physics Letters 86, 023508 (2005).
Hoffman, J., Shao, J., Hsu, C.-H., and Folch, A. “Elastomeric Molds with Tunable Microtopographies”, Advanced Materials 16:2201 (2004).
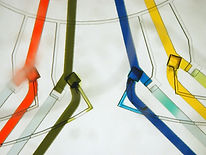
Gradient Generators
Biomolecule gradients have been shown to play roles in a wide range of biological processes including development, inflammation, wound healing, and cancer metastasis. Elucidation of these phenomena requires the ability to expose cells to biomolecule gradients that are quantifiable, controllable, and mimic those that are present in vivo. Microfluidic gradient generators offer greater levels of precision, quantitation, and spatiotemporal gradient control than traditional methods, and may greatly enhance our understanding of many biological phenomena.
References:
C. G. Sip, N. Bhattacharjee, and A. Folch, “A Modular Cell Culture Device for Generating Arrays of Gradients Using Stacked Microfluidic Flows”, Biomicrofluidics 5, 022210 (2011).
D. M. Cate, C. G. Sip, and A. Folch, "A microfluidic platform for generation of sharp gradients in open-access culture", Biomicrofluidics 4, 044105 (2010).
Keenan, T.M., Frevert, C.W., Wu, A., Wong, V., and Folch, A. “A New Method for Studying Gradient-Induced Neutrophil Desensitization Based on an Open Microfluidic Chamber”, Lab Chip 10: 116 (2010).
Bhattacharjee, N., Li, N., Keenan, T.M., and Folch, A. “A Neuron-Benign Microfluidic Gradient Generator for Studying the Growth of Mammalian Neurons towards Axon Guidance Factors”, Integrative Biology 2, 669 (2010).
Keenan, T.M. and Folch, A. “Biomolecular gradients in cell culture systems”, Lab Chip 8, 34 (2008).
Hsu, C.-H. and Folch, A. “Spatiotemporally-Complex Concentration Profiles Using a Tunable Chaotic Micromixer”, Appl. Phys. Lett. 89: 144102 (2006).
Neils, C. M., Tyree, Z., Finlayson, B., and Folch, A. “Combinatorial Mixing of Microfluidic Streams”, Lab on a Chip 4:342 (2004).
Chen, C., Hirdes, D., and Folch, A. “Gray-Scale Photolithography Using Microfluidic Photomasks”, Proceedings of National Academy of Sciences 100:1499 (2003).
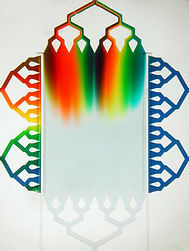